Catheter ablation for medication-refractory scar-related ventricular tachycardia (VT) was first performed in 1983, inspired by earlier VT procedures in which endocardial arrhythmogenic substrate was mapped and surgically resected.1,2 VT ablation became more common in the late 1980s with the advent of radiofrequency energy ablation, which enhanced procedural safety compared with its unipolar direct current predecessor. Around this time, activation and entrainment mapping emerged as the dominant strategies for localising key areas of ventricular myocardium participating in the macroreentrant circuit(s) underlying the majority of scar-related VT.3 Both strategies rely on the presence of either spontaneous or induced VT, and these tools continue to be widely used through the present day.
With activation mapping, a mapping catheter is moved around the ventricular endocardium during VT to record the timing of local electrograms relative to the 12-lead ECG, to determine the sequence of ventricular activation. Adjacent areas exhibiting electrical potentials during ventricular diastole are considered likely to participate in the ‘common channel’ or ‘critical isthmus’ of the re-entrant circuit, and thus are targeted for ablation. Entrainment mapping entails pacing the ventricle at a cycle length slightly shorter than that of the VT. Sites at which this pacing continuously resets the VT with concealed fusion, and at which the return cycle after the cessation of pacing is equal to the native tachycardia cycle length, are deduced to be critical areas of the VT circuit (Figure 1A).4
The Problem of Haemodynamic Instability
The initial years of VT ablation predated the widespread adoption of either percutaneous coronary intervention or neurohormonal blockade following MI. For this reason, most scar-related VT was found in post-MI patients with dense, confluent scars that engendered VT of sufficiently long cycle lengths to be haemodynamically tolerated during activation or entrainment mapping. In recent decades, however, advances in post-MI care have resulted in many patients having left ventricular scars that are smaller, less dense and more heterogeneous, interspersed with surviving cardiomyocytes. This evolution of post-MI scar architecture, as well as the selection of patients for ablation with more advanced heart failure or comorbid illnesses, have led to VTs that are, on average, faster and/or haemodynamically less well tolerated.5 It was initially hypothesised that these faster VTs must reflect smaller VT re-entrant circuits. However, Nishimura et al. recently challenged this assumption by demonstrating no difference in median circuit length between 24 fast (cycle length <333 ms) and 30 slow (cycle length >333 ms) scar-related VTs in a cohort of 49 patients undergoing ablation. Rather than circuit dimensions, they found that conduction velocity in the outer loop was the most significant determinant of VT cycle length, both in ischaemic cardiomyopathy (ICM; r=–0.5, p=0.006) and non-ICM (NICM; r=-0.45, p=0.028).6
In a historical context, it seems likely that more effectively treated MIs have led to VT circuits with outer loops that contain sparser fibrosis, thereby facilitating higher propagation velocities and shorter VT cycle lengths. As a result, it is estimated that now only ~30% of patients referred for scar-related VT can be safely ablated using exclusively activation and entrainment mapping during VT.7 Among 231 participants in the observational Thermocool VT ablation trial, for example, 69% had unmappable VTs – 78% of which were haemodynamically unstable, including two patient deaths from incessant VT during the procedure.8
In patients with structural heart disease undergoing VT ablation, haemodynamic derangements may persist even after successful cardioversion of an induced unstable VT. Skhirtladze et al. demonstrated this concept by invasively measuring cardiac output in 63 patients undergoing ICD placement for secondary prevention of cardiac arrest. Ventricular fibrillation or pulseless VT was induced for 10 seconds during ICD threshold testing, followed by internal defibrillation, after which the time to recovery of baseline cardiac output was documented for each patient. They found that systolic dysfunction correlated with time to recovery after defibrillation: those with left ventricular ejection fraction (LVEF) <30% took a median of 17 seconds to regain baseline cardiac output, compared with 14 and 0 seconds in those with LVEF 30–50% and >50%, respectively (p<0.05).9
This phenomenon of delayed haemodynamic recovery was similarly observed by Miller et al. in their PERMIT1 study. In PERMIT1, 20 patients with LVEF ≤40% underwent VT ablation with mechanical support from the Impella 2.5 percutaneous left ventricular assist device (pLVAD), using non-invasive cerebral oximetry as a metric of haemodynamic status. Despite haemodynamic support from intravenous phenylephrine and pLVADs, 17% of the induced unstable VTs precipitated cerebral oxygen desaturations <55%, requiring cardioversion. Even when the cerebral oxygen saturation remained >55% for one or more consecutive VT episodes, the investigators observed a gradual decrease in cerebral oxygen saturation with repeated VT inductions over the course of a several-hour case, reflecting delayed haemodynamic recovery (Supplementary Materials Figure 1).10

Furthermore, haemodynamic instability during VT ablation may adversely affect both the short- and long-term prognosis (Figure 2). In a Medicare claims analysis of 345 patients who underwent VT ablation, Aryana et al. showed that a higher level of invasive haemodynamic support during the procedure (pLVAD versus intra-aortic balloon pump) was associated with lower rates of in-hospital renal failure (11.7% versus 21.7%), in-hospital mortality (6.5% versus 19.1%) and 30-day rehospitalisation (27% versus 38.7%, all p<0.05), despite a higher baseline prevalence of chronic kidney disease and heart failure in the pLVAD group.11
Kuo et al. found periprocedural haemodynamic decompensation to be independently predictive of both 30-day mortality (HR 5.13; p=0.02) and 1-year mortality (HR 2.59; p=0.05) in a retrospective study of 317 patients undergoing scar-related VT ablation. Despite its borderline statistical significance, the association between haemodynamic decompensation and 1-year mortality is made more convincing by the fact that haemodynamic decompensation independently predicted acute kidney injury (OR 3.98; p=0.03), and acute kidney injury in turn predicted 1-year mortality (HR 3.28; p=0.005).12 In a similar vein, Santangeli et al. identified the presence of unmappable VTs as an independent predictor of 1-month mortality (OR 5.69; p=0.017) in a large international cohort of 2,061 patients undergoing ablation for scar-related VT.13 Given their inherent risk of confounding, these observational studies should be viewed as exploratory rather than definitive.
Mechanical Circulatory Support
As alluded to above, at some institutions the challenge of mapping haemodynamically unstable VTs is addressed by the selective use of pLVADs during VT ablation. Mariani et al. performed a meta-analysis of five observational studies encompassing 394 patients (55% with ICM) undergoing ablation of haemodynamically unstable VT with or without prophylactic implantation of a pLVAD (86.6% Impella, 13.4% TandemHeart).14 Baseline characteristics, including mean LVEF and prevalence of chronic kidney disease, class III/IV heart failure and electrical storm, were similar between groups. VT induction with activation and entrainment mapping was the primary ablation strategy employed for all patients. The authors found that prophylactic pLVAD use facilitated a mean of 24 additional minutes of VT mapping per patient. Despite similar rates of post-procedure VT inducibility and long-term VT recurrence between groups, prophylactic pLVAD use was associated with lower mortality at 3–19 months of follow-up (OR 0.55; 95% [CI 0.32–0.94]) and a non-significantly lower in-hospital or 30-day mortality (OR 0.55; 95% CI [0.28–1.05]). Without an improvement in VT outcomes, the improved mortality may be inferred to be due to the maintenance of procedural haemodynamic stability rather than superior ablation efficacy with prophylactic pLVAD use.
Importantly, the use of pLVADs carries inherent additional risk of vascular injury and bleeding complications related to endovascular device placement and more intensive anticoagulation, respectively.14 Widespread use of pLVADs is also limited by cost. To risk-stratify patients who could benefit most from prophylactic pLVAD placement, Santangeli et al. developed the PAINESD risk score based on univariate predictors of periprocedural haemodynamic decompensation in a cohort of 193 patients undergoing scar-related VT ablation.15 Reflected in the scoring system (ranging from 0 to 35 points), the authors found that the presence of chronic obstructive pulmonary disease, age >60 years, general anaesthesia use, ICM, New York Heart Association class III or IV heart failure, LVEF <25%, VT storm, or diabetes conferred incremental risk of haemodynamic decompensation. The PAINESD score was validated in a separate propensity-matched analysis of 150 patients undergoing scar-related VT ablation with or without prophylactic pLVAD use, which showed a substantial mortality benefit in patients with PAINESD ≥15 (HR 0.43; 95% CI [0.21–0.87]), but none in patients with PAINESD ≤8 (HR 0.63; 95% CI [0.24–1.66]).16
Ablating Without Inducing: A Historical Precedent
The prospect of avoiding VT induction altogether during VT ablation is appealing, to maintain haemodynamic stability while avoiding the excess cost and risks of invasive mechanical support. The precedent for effective invasive VT management in the absence of VT mapping is not new. Sosa et al. demonstrated a 5-year VT recurrence rate of just 12% among 50 consecutive patients with sustained VT related to post-MI anteroseptal aneurysms who were referred for surgical left ventricular reconstruction between 1986 and 1994.17 The surgery involved aneurysmectomy and septal plication using a double-plicature technique, but with no intra-operative VT mapping. VT was inducible in all patients preoperatively, but only 4% of patients postoperatively. Patients experienced a mean absolute LVEF improvement of 12%.
Mortality was high among these patients (27% at 5 years), despite improved systolic function and low rates of VT recurrence, which likely reflected progressive heart failure and multiple comorbidities (Supplementary Materials Figure 2).17 Participants in this study did not have ICDs, which may have led to an underestimation of VT recurrence. Furthermore, the apparent success of surgical left ventricular reconstruction in these patients, all of whom had dense anterior MIs from left anterior descending coronary artery occlusion, is not necessarily generalisable to patients with more heterogeneous VT substrate. Nonetheless, the authors’ observations provided early evidence that extensive modification of VT substrate can successfully eliminate scar-related VT without requiring concomitant VT mapping.
Substrate Mapping
The advent of magnetic electroanatomical mapping systems in the late 1990s facilitated the development of substrate mapping – a family of catheter-based techniques employed to localise critical VT circuit components during sinus or paced rhythm.18 These techniques can conceptually overlap, and more than one technique is often used to modify the potential VT substrate.
Pace Mapping
The 1980s saw the emergence of a precursor to substrate mapping known as pace-mapping, a technique wherein a catheter is used to pace the ventricular endocardium at various locations. When pacing from one or more points generates a 12-lead ECG whose morphology matches that of the clinical VT, those points are considered to be close to the critical components of the VT circuit, such as an exit site for re-entrant VT or a source of origin for a focal VT (Figure 1B). Spatial resolution of this technique can be low, however, with ECG-matched pace-maps observed as far as 2.6 cm from the VT’s site of origin. The latter phenomenon can be seen when pacing an area of scar outside the VT circuit leads to depolarisation of the rest of the ventricle in a sequence resembling that of the clinical VT.
Conversely, pace-mapping may lack sensitivity for critical VT circuit components other than isthmus exit sites, as distinct ECG morphologies often result from pacing at an isthmus entrance site.18 Furthermore, pace-mapping does not necessarily obviate or safeguard against VT induction. If the clinical VT was not captured on 12-lead ECG prior to ablation, it must be induced to compare it with the pace maps. In addition, pace-mapping may unintentionally precipitate VT – indeed, sites of so-called ‘pace-mapped induction’ often signify areas where ablation will terminate the VT.19
Voltage Mapping
The earliest form of electroanatomically-based substrate mapping was voltage mapping, first described by Marchlinski et al. in a cohort of 16 patients undergoing ablation for unstable VT in a background of ICM or NICM. Guided by prior intraoperative mapping experience, as well as measurements obtained in a control group of patients without structural heart disease, the authors considered a peak-to-peak local electrogram amplitude >1.5 mV to represent normal endocardium, 0.5–1.5 mV to represent scar ‘border zones’ and <0.5 mV to represent dense scar. These endocardial regions were readily distinguishable in a colour-coded model generated by an electroanatomical mapping system. The authors ablated in linear patterns connecting areas of dense scar to normal endocardium, thus transecting scar border zones suspected of harbouring critical components of the VT circuit.20 This ablation strategy resulted in a 25% VT recurrence rate at 8 months of follow-up.
While still widely used, voltage mapping has important limitations. Because the bipolar amplitude of local electrograms varies with electrode size and interelectrode spacing, the voltage thresholds used to define scar cannot be universally standardised, and must be adjusted when using newer, higher-density mapping catheters.21 Furthermore, a bipolar voltage map is dependent on the wave front of pacing used and how that wave front activates the scar (Figure 3).

Tung et al. demonstrated that local endocardial voltage is influenced by the wave front of activation (e.g. sinus rhythm versus RV pacing), and that 18% of VT isthmuses may fall in areas of ‘normal’ voltage if only one wave front is observed.22 As discussed by Wiles et al., omnipolar voltage mapping may circumvent this issue, although clinical experience in humans remains limited.4 Finally, bipolar voltage mapping alone is relatively non-specific for VT isthmuses and, therefore, may lead to excessive ablation, particularly in patients with large areas of scar. Anter et al. showed in swine models of infarct-related VT that VT isthmuses occupied only 18% of the low-voltage endocardium (<1.5 mV), and that low voltage was 72% sensitive, but only 56% specific for VT isthmuses.23
Local Electrogram Morphology and Timing
The next 15 years saw the emergence of a second wave of substrate mapping techniques, this time focused on the morphology and timing, rather than amplitude, of local electrograms during sinus or paced rhythm. Abnormal local electrogram morphologies can be categorised as fractionated, split, isolated late and local abnormal ventricular activity.23 These ablation techniques include:
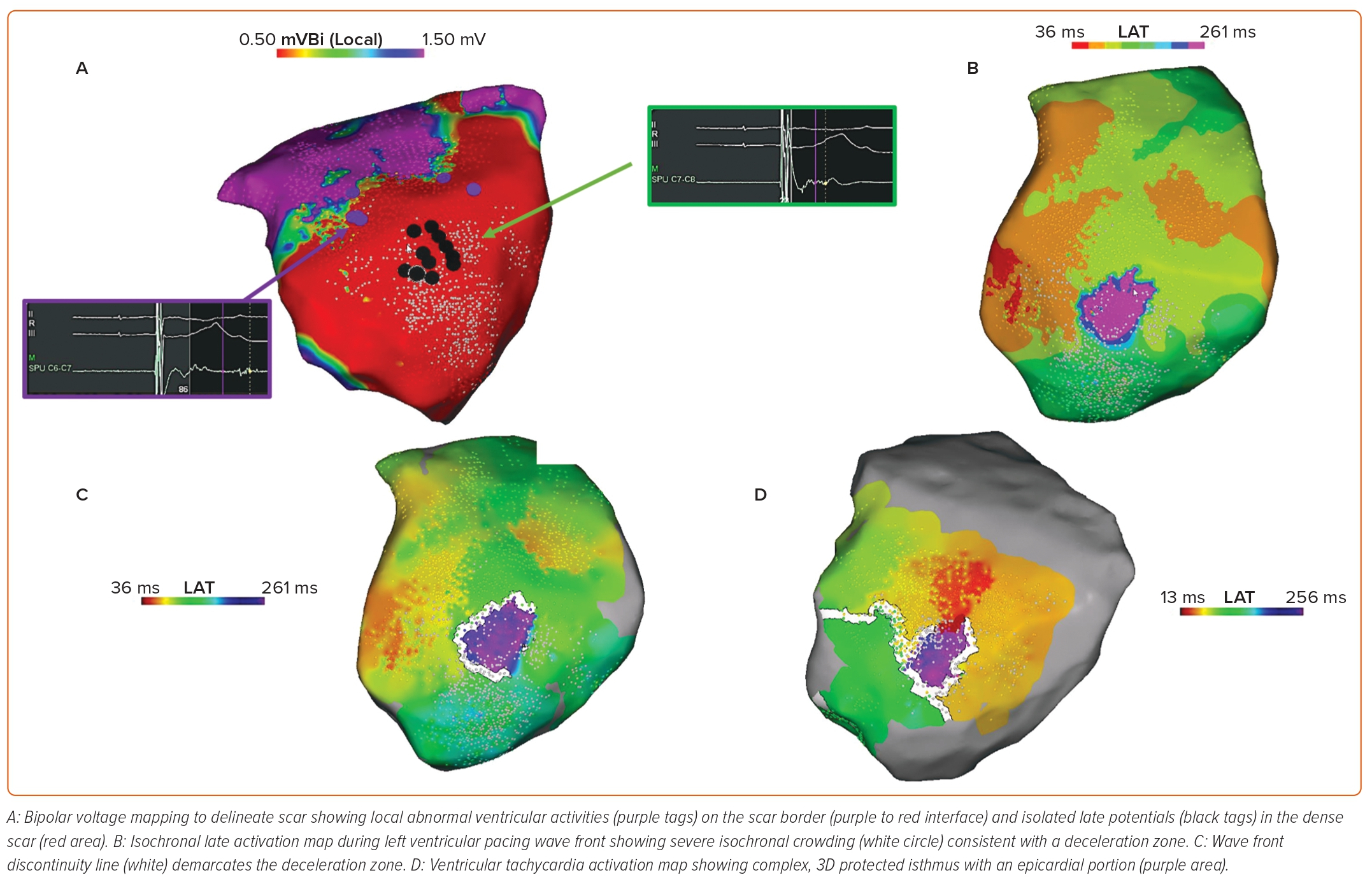
- Late potential ablation – Late potentials were defined by Arenal et al. as electrograms showing double or multiple components separated by >50 ms of an isoelectric interval or very low amplitude signals (Figure 4A). The underlying rationale was that isolated electrogram components occurring late with respect to the surface QRS complex represent areas of slowed conduction and may be analogous to diastolic potentials during VT activation mapping – denoting the presence of a slow-conducting isthmus of a re-entrant VT circuit.24
- Local abnormal ventricular activity ablation – Jaïs et al. developed a more selective approach to substrate homogenisation, targeting a narrower subset of abnormal electrograms that they termed ‘local abnormal ventricular activities’ (LAVAs). LAVAs were defined as sharp, high-frequency signals occurring in sinus rhythm anytime during or after the far-field ventricular electrogram, which exhibited poor coupling to the rest of the myocardium with pacing manoeuvres (Figure 4A).25
- Scar homogenisation – A more liberal and probabilistic approach known as scar homogenisation was demonstrated by Di Biase et al. for the treatment of 43 patients with ICM presenting in VT storm. The investigators performed both endocardial and epicardial voltage mapping on all patients, and ablated any abnormal electrograms, defined as electrograms <1.5 mV in amplitude, >70 ms in duration and containing more than three deflections. While this strategy can lead to similar or better results than traditional activation/entrainment map, scar homogenisation requires extensive ablation, resulting in longer procedures, fluoroscopy time and radiofrequency time.26
- Scar dechannelling – The technique of scar dechannelling is based on the principle that ‘conduction channels’ identified during sinus or paced rhythm may correspond to critical VT circuit components. Arenal et al. initially demonstrated this with high-density voltage mapping and defined conduction channels as linear regions with relatively preserved voltage surrounded on either side by lower-voltage endocardium.27 Building upon this approach, Tung et al. redefined conduction channels as contiguous areas of slow conduction exhibiting a gradient of activation delay. By ablating just the earliest subset of late potentials harboured in the entrances to these channels, they were able to block conduction more distally and achieve a low rate of VT recurrence.28
- Core isolation – In 2015, Tzou et al. described a novel ablation strategy for scar-related VT known as ‘core isolation’, where confirmed VT isthmuses or potential VT substrate areas (identified with voltage mapping, pace-mapping and amplitude-based conduction channels) were surrounded by a circumferential ablation lesion set. Isolation of the arrhythmogenic tissue was confirmed by exit block by pacing inside the ablated perimeter.29
Each of these techniques has limited specificity, as abnormal potentials can be seen in areas of scar or dead-end pathways during VT that do not participate in a clinical VT. Consequently, contemporary approaches to VT substrate ablation should ideally also incorporate ‘functional substrate mapping’ – a group of techniques aimed at delineating the dynamic, rather than static, electrical properties of the myocardium.
Functional Substrate Mapping: Isochronal Late Activation Mapping and Wave Front
Discontinuities
With the goal of enhancing the specificity of substrate mapping for critical VT circuit components, Irie et al. were the first to demonstrate the technique of isochronal late activation mapping (ILAM) in humans.30 The investigators retrospectively analysed 33 patients who had undergone scar-related VT ablation with activation and entrainment mapping, and harboured a total of 47 VT ‘critical sites’, defined as myocardial areas in or adjacent to low-voltage regions that exhibited diastolic activity during VT and resulted in VT termination when ablated. To narrow down which abnormal electrograms during sinus or paced rhythm correlated with VT critical sites, they stratified local electrograms by timing of completion of activation (i.e. offset of the latest bipolar deflection). This information was then used to construct a colour-coded ventricular map comprising eight sequential isochrones – each corresponding to 12.5% of the ventricular activation window (Figure 4B). Interestingly, the majority of critical sites (64%) were found in the second- and third-latest isochrones (62.5–87.5% of the ventricular activation window), with only 11% in the latest isochrone (87.5–100% of the ventricular activation window). Furthermore, a median of three isochrones were found within a 1 cm radius of critical zones – a phenomenon the authors termed ‘isochronal crowding’, which signifies regions of electrical wave front deceleration (also called ‘deceleration zones’).30 Deceleration zones appear to retain a fixed position irrespective of the wave front of ventricular activation, despite differences in isochronal sequence (Figure 3).
Newer electroanatomic mapping systems can reconstruct sinus or paced rhythm activation by automatically annotating the latest nearfield electrogram, and wave front discontinuity lines representing lines of conduction block or delay can be automatically identified and targeted with ablation (Figure 4C). Wave front discontinuity lines during sinus or paced rhythm can closely correlate with lateral boundaries and protected isthmuses during VT (Figure 4D). Limiting the ablation of abnormal ventricular potentials to regions of isochronal crowding or wave front discontinuity lines offers a more focused approach than previous substrate ablation methods, and apparently without sacrificing sensitivity for VT critical sites or clinical efficacy. In a more recent prospective study of 120 patients undergoing VT ablation using ILAM, ablated regions constituted only 18% of the total bipolar low-voltage area, 95% of inducible VT critical sites occurred in areas of isochronal crowding and 80% of patients were free of VT recurrence at 12 months.31
Advanced Cardiac Imaging
In recent years, preprocedural cardiac CT angiography (CTA) as well as MRI – when not precluded by an MRI-incompatible cardiac implantable electrical device or generator-associated artefact – have become part of the standard of care for planning substrate ablation of scar-related VT. In a cohort of 116 patients undergoing ablation of VT related to ischaemic, non-ischaemic or right ventricular arrhythmogenic cardiomyopathy, Yamashita et al. found that 85% of LAVAs mapped to regions of ventricular wall-thinning or hypoattenuation on CTA, or late gadolinium enhancement (LGE) on MRI (collectively referred to as ‘imaging substrate’).32 Hence, focusing electroanatomical mapping to myocardial areas containing imaging substrate could potentially shorten procedure times. Furthermore, LGE on cardiac MRI or late iodine enhancement on cardiac CTA may identify intramural substrate that conventional voltage mapping would not detect.
The presence of epicardial imaging substrate influenced Yamashita et al.’s decision to obtain epicardial access in 33% of their patients. Moreover, the investigators modified their epicardial ablation strategy in 76% of patients based upon the proximity of coronary arteries or phrenic nerves visualised on cardiac CT or MRI and integrated into the electroanatomic mapping system. Direct visualisation of these structures obviated coronary angiography or phrenic nerve pacing in many cases.32 Takigawa et al. later described the cardiac CTA finding of ‘wall thickness channels’ (WTCs) in nine patients with ICM undergoing VT ablation with a combination of activation/entrainment mapping and LAVA elimination. WTCs referred to ridges of thin ventricular myocardium (<5 mm) surrounded on either side by even thinner myocardium, and were hypothesised to contain heterogeneous scars with surviving cardiomyocyte bundles capable of forming VT isthmuses. The authors indeed found that while only 51% of WTCs colocalised with a VT isthmus, all 10 VT isthmuses colocalised with one of more WTC.33 Data from our own institution suggest that while LGE, late iodine enhancement and wall-thinning are highly sensitive for deceleration zones during ILAM, WTCs have a relatively low sensitivity and specificity for this substrate feature.
Finally, Andreu et al. demonstrated that high-resolution cardiac MRI can enhance the effectiveness of traditional scar dechannelling.34 The investigators prospectively evaluated a cohort of 159 patients with scar-related VT who underwent substrate ablation using scar dechannelling, wherein conducting channels were identified by the varying precocity of delayed components of local electrograms, and channel entrances (marked by the earliest delayed components) were ablated. They performed preprocedural cardiac MRI on 54 of these patients, and constructed maps of pixel signal intensity based upon the resulting LGE distribution. In turn, pixel signal intensity maps were used to define ‘heterogeneous tissue channels’ – linear areas of moderate signal intensity (representing scar border zones) connecting two regions of normal signal intensity and surrounded on either side by higher signal intensity (representing dense scar). Seventy-seven per cent of conducting channels colocalised with heterogeneous tissue channels when cardiac MRIs for these 54 patients were coregistered to their electroanatomical maps. Qualitatively, the authors observed that MRI-derived heterogeneous tissue channels helped both to focus electroanatomical mapping to high-yield myocardial regions, as well as to clarify the geometry of otherwise ambiguous conducting channels. These advantages translated to a lower radiofrequency time (19 ± 12 minutes versus 27 ± 16 minutes; p=0.009), post-ablation VT inducibility rate (32% versus 51%; p=0.022) and 20-month VT recurrence rate in the MRI-guided ablation cohort (18.5% versus 43.8%; p=0.017).
Clinical Efficacy of Substrate Versus Conventional VT Ablation
In addition to mitigating the risk of periprocedural haemodynamic decompensation, substrate ablation for scar-related VT has also demonstrated promising long-term clinical outcomes. In a prospective multicentre registry of 412 patients undergoing VT substrate ablation using a scar dechannelling technique, 1-year VT-free survival was 82.5%.35 Di Biase et al. performed the first randomised controlled trial comparing substrate with conventional VT ablation in 118 patients with ICM: the VISTA trial.36 Patients in the conventional ablation group underwent VT induction with a combination of activation, entrainment and pace-mapping targeting clinical VTs, as well as any haemodynamically tolerated non-clinical VTs; patients in the substrate ablation group underwent scar homogenisation without VT induction. Impressively, patients randomised to substrate ablation experienced lower rates of mortality or rehospitalisation (20.7% versus 46.7%; p=0.003), as well as VT recurrence (15.5% versus 48.3%, p<0.001) at 1 year of follow-up. The lower VT recurrence rate with substrate ablation in this study may be understood within a pathophysiologic framework highlighted by Anter et al.23 Different VTs within the same patient rely on critical sites, which, although often sharing isthmuses in common, can nonetheless be spatially distinct from one another. Conventional VT mapping can identify critical sites underlying inducible, stable VTs, but these may represent only a fraction of the potential VTs supported by a patient’s scar geometry. Substrate mapping, by contrast, has the potential to identify any VT critical site that is manifest through conduction slowing or local electrogram abnormalities. The lower mortality and rehospitalisation rate seen in the substrate ablation group may reflect fewer haemodynamic perturbations, as well as fewer VT recurrences.
In a smaller randomised trial of 48 patients (including 10 with NICM), Fernández-Armenta et al. compared two hybrid ablation strategies with each other. In the experimental group, substrate ablation using scar dechannelling was performed first, followed by activation and entrainment mapping of any VTs that could be subsequently induced. Patients in the control group underwent VT induction with activation and entrainment mapping first, followed by scar dechannelling. Given the use of conventional and substrate-based VT mapping in both experimental and control groups, it is not surprising that the authors found similar rates of VT recurrence at 22 months of follow-up (41.7% versus 33.3%; p=0.56). Importantly, however, performing substrate ablation before attempting VT induction resulted in fewer electrical cardioversions (25% versus 54%; p=0.039), and shorter mean procedure time (209 versus 262 minutes; p=0.009) and fluoroscopy time (14 versus 21 minutes; p=0.005).37 Electrical cardioversions may precipitate cardioembolic events; while statistically underpowered, it is nonetheless worthwhile to note that the one transient ischaemic attack in this cohort occurred following the cardioversion of an unstable VT induced in the control group. The ability of a ‘substrate-first’ ablation strategy to shorten procedure times is also meaningful, given that procedure time has been independently associated with in-hospital mortality.38 Finally, reducing fluoroscopy-associated radiation exposure is desirable for the safety of both patients and physicians.
Two meta-analyses also compared conventional with substrate-based VT ablation with respect to long-term clinical outcomes.39,40 The analyses were designed similarly – each including one randomised controlled trial and six observational studies with a total of ~400 patients.36 Substrate ablation techniques included pace-mapping, late potential ablation and scar homogenisation. Both meta-analyses identified non-significant trends of lower VT recurrence (Kumar RR 0.72; 95% CI [0.44–1.18]; Briceño RR 0.68; 95% CI [0.40–1.15]) and all-cause mortality (Kumar RR 0.76; 95% CI [0.36–1.59]; Briceño RR 0.56; 95% CI [0.29–1.09]) with substrate compared with conventional VT ablation at 18–24 months of follow-up. Briceño et al. demonstrated that the composite of VT recurrence or all-cause mortality occurred less frequently in the substrate ablation group (RR 0.57; 95% CI [0.40–0.81]). Of note, both meta-analyses included several non-randomised studies in which substrate ablation was used only for patients with haemodynamically unstable clinical VT, which one would expect to bias the results towards an overestimation of mortality in the substrate ablation group. Recognising this bias makes Briceño et al.’s conclusion of substrate ablation’s superiority all the more persuasive.
To our knowledge, Bunch et al. conducted the only study date comparing pLVAD-supported conventional VT mapping with substrate mapping for the ablation of unstable scar-related VT.41 The investigators compared 13 patients undergoing activation and entrainment mapping with prophylactic TandemHeart placement with 18 patients with matched baseline characteristics undergoing substrate ablation using a combination of abnormal local electrograms and pace-mapping. The mean procedure time was longer in the pLVAD/conventional mapping group (400 versus 274 minutes; p=0.001). There was no difference in the rate of procedural complications between conventional and substrate ablation groups, and VT-free survival at 9 months was similar (55% versus 48%; p=0.96).

Substrate Ablation with Selective VT Induction: A Tailored Approach
VT substrate mapping and activation/entrainment mapping may be complementary to each other, and therefore both desirable, when a clinical VT is haemodynamically tolerated. We therefore propose a tailored approach to scar-related VT ablation that accounts for variability in both the risk of haemodynamic deterioration and the probability of identifying adequate VT substrate (Figure 5). In this algorithm, we consider a PAINESD score ≥15, 9–14 and ≤8 to represent high, intermediate and low risk of haemodynamic decompensation, respectively.
For patients with ICM at high haemodynamic risk, we avoid VT induction altogether, relying exclusively on substrate mapping. For ICM patients at intermediate or low haemodynamic risk, we induce VT selectively when VT substrate cannot be adequately identified and/or modified during sinus or paced rhythm. We employ this selective VT induction strategy in both intermediate- and high-risk patients with NICM, given that VT substrate tends to be sparser and more challenging to identify in this population. In patients with NICM and low haemodynamic risk, we perform routine VT induction following substrate mapping to maximise the likelihood of successfully ablating all VT critical sites.
If VT recurrence prompts a second ablation attempt, VT induction should be considered for patients of all risk strata, particularly if there is insufficient remaining modifiable substrate. This should be undertaken with consideration of mechanical circulatory support for patients with a PAINESD score ≥15. Epicardial mapping should be considered if not performed during the initial ablation – particularly for patients with NICM, a 12-lead ECG suggestive of an epicardial VT critical site, or cross-sectional imaging demonstrating epicardial LGE or late iodine enhancement. At this point, operators may also consider ‘bail-out’ techniques targeting mid-myocardial substrate, such as bipolar ablation or low ionic irrigant ablation to generate larger ablation lesions.4,42
VT substrate mapping can include any of the above-mentioned techniques; however, for most patients, we prefer a functional substrate mapping approach using ILAM or targeting wave front discontinuity lines defined in at least one wave front. Due to the wave front dependence of bipolar electrogram voltage and morphology, an apparent lack of functional substrate in one wave front should prompt re-mapping in a second wave front (sinus rhythm, RV paced rhythm or LV paced rhythm), where deceleration zones or wave front discontinuities may be better appreciated.
Patients at high haemodynamic risk may not tolerate prolonged substrate mapping under general anaesthesia; in this high-risk subgroup, we therefore recommend focused mapping strategies (e.g. ILAM, preprocedural CT/MRI) rather than scar homogenisation. Mapping of spontaneous VT, if haemodynamically tolerated, may be attempted briefly, even in high-risk patients. Patients with NICM often have mid-myocardial substrate visualised by cardiac CT or MRI, but not by endocardial or epicardial surface mapping. Coregistration of CT/MRI-based scar segmentations with electroanatomical maps often facilitates successful ablation of these regions.
Conclusion
In response to the increasing prevalence of fast, haemodynamically unstable VT associated with advancements in the treatment of MI, several substrate mapping techniques have been developed to localise VT circuits without inducing VT. Over time, the focus of these techniques has shifted from local electrogram amplitude in voltage mapping, to electrogram morphology and timing in late potential ablation, scar homogenisation, and scar dechannelling, to zones of conduction deceleration in isochronal late activation mapping, and, finally, to the integration of non-invasive imaging data with electroanatomical mapping. Two meta-analyses and two small randomised trials suggest that substrate-based approaches offer superior clinical outcomes to conventional ablation with VT induction.
Little is known about the comparative efficacy of different substrate ablation techniques, and this warrants further research. Conventional VT mapping with mechanical circulatory support showed comparable outcomes with substrate mapping in one study, but additional head-to-head comparisons are required, along with cost-effectiveness analyses. As substrate mapping techniques continue to improve, ablating scar-related VT without any VT induction may become increasingly reliable and effective – thus avoiding periprocedural haemodynamic decompensation, and its associated morbidity and mortality in patients at highest risk.
Click here to view Supplementary Material
Clinical perspectives
- Traditional approaches to ventricular tachycardia (VT) ablation that rely on inducing VT often precipitate haemodynamic compromise, which is associated with increased mortality.
- ‘Substrate modification’ techniques use local electrogram features to identify VT isthmuses during sinus or paced rhythm, thus minimising the need for VT induction.
- Randomised data are sparse, but suggest lower mortality and VT recurrence rates with substrate modification compared with traditional approaches reliant on VT induction.
- VT induction with temporary mechanical circulatory support may offer similar haemodynamic protection to substrate modification, but is likely less cost effective.
- A tailored approach combining substrate modification with selective VT induction can be useful in clinical practice, guided by the PAINESD risk score for periprocedural haemodynamic compromise.